Impact of Oxidative Stress
Dr. Bryan D. McKersie, University of Guelph (Posted on the Internet in 1996)
ACTIVATION OF OXYGEN
One of the paradoxes of life on this planet is that the molecule that sustains aerobic life, oxygen, is not only fundamentally essential for energy metabolism and respiration, but it has been implicated in many diseases and degenerative conditions (Marx, 1985). A common element in such diverse human disorders as ageing, arthritis, cancer, Lou Gehrig’s disease and many others is the involvement of partially reduced forms of oxygen. Our realisation of the significance of oxygen in disorders and stress-induced dysfunctions in cultivated plants is recent due in no small part to the difficulty in detecting and tracing oxygen molecules, to the multitude of forms and intermediates that oxygen can assume, and to the extreme reactivity and rate of the chemical reactions involved. As a consequence, we often in our experiments can only look for the “footprints” of oxygen reactions in our attempts to determine cause-effect relationships in stress responses. The following chapter describes our current understanding of the general principles of activated oxygen.
Atmospheric oxygen in its ground-state is distinctive among the gaseous elements because it is a biradical, or in other words it has two unpaired electrons. This feature makes oxygen paramagnetic; it also makes oxygen very unlikely to participate in reactions with organic molecules unless it is “activated”. The requirement for activation occurs because the two unpaired electrons in oxygen have parallel spins. According to Pauli’s exclusion principle, this precludes reactions with a divalent reductant, unless this reductant also has two unpaired electrons with parallel spin opposite to that of the oxygen, which is a very rare occurrence. Hence, oxygen is usually non-reactive to organic molecules which have paired electrons with opposite spins. This spin restriction means that the most common mechanisms of oxygen reduction in biochemical reactions are those involving transfer of only a single electron (monovalent reduction).
Activation of oxygen may occur by two different mechanisms: absorption of sufficient energy to reverse the spin on one of the unpaired electrons, or monovalent reduction. The biradical form of oxygen is in a triplet ground state because the electrons have parallel spins. If triplet oxygen absorbs sufficient energy to reverse the spin of one of its unpaired electrons, it will form the singlet state, in which the two electrons have opposite spins (Fig. 1). This activation overcomes the spin restriction and singlet oxygen can consequently participate in reactions involving the simultaneous transfer of two electrons (divalent reduction). Since paired electrons are common in organic molecules, singlet oxygen is much more reactive towards organic molecules than its triplet counterpart.
The second mechanism of activation is by the stepwise monovalent reduction of oxygen to form superoxide (O 2), hydrogen peroxide (H2O2), hydroxyl radical (*OH) and finally water according to the scheme shown in figure 2. The first step in the reduction of oxygen forming superoxide is endothermic but subsequent reductions are exothermic.
Superoxide can act as either an oxidant or a reductant; it can oxidise sulphur, ascorbic acid or NADPH; it can reduce cytochrome C and metal ions. A dismutation reaction leading to the formation of hydrogen peroxide and oxygen can occur spontaneously or is catalysed by the enzyme superoxide dismutase. In its protonated form (pKa = 4.8) superoxide forms the perhydroxyl radical (*OOH) which is a powerful oxidant (Gebicki and Bielski, 1981), but its biological relevance is probably minor because of its low concentration at physiological pH.
The univalent reduction of superoxide produces hydrogen peroxide which is not a free radical because all of its electrons are paired (Fig. 2). Very often the reduction products of oxygen are referred to by biologists as oxygen free radicals which is a misnomer because in chemistry a free radical is defined as an atom or molecule with an unpaired electron. It is more appropriate to refer to the intermediate reduction products of oxygen as activated not as free radicals because triplet oxygen (ground state) is a radical and hydrogen peroxide is not.
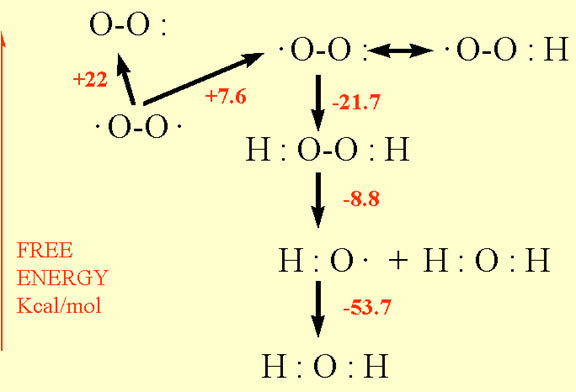
Hydrogen peroxide is noteworthy because it readily permeates membranes and it is therefore not compartmentalised in the cell. Numerous enzymes (peroxidases) use hydrogen peroxide as a substrate in oxidation reactions involving the synthesis of complex organic molecules. The well-known reactivity of hydrogen peroxide is not due to its reactivity per se, but requires the presence of a metal reductant to form the highly reactive hydroxyl radical which is the strongest oxidizing agent known and reacts with organic molecules at diffusion-limited rates.
Fenton described in the late nineteenth century (Fenton, 1894; 1899) the oxidising potential of hydrogen peroxide mixed with ferrous salts. Forty years later, Haber and Weiss (1934) identified the hydroxyl radical as the oxidising species in these reactions:
In biological systems the availability of ferrous ions limits the rate of reaction, but the recycling of iron from the ferric to the ferrous form by a reducing agent can maintain an ongoing Fenton reaction leading to the generation of hydroxyl radicals. One suitable reducing agent is superoxide which participates in the overall reaction 2 as two half reactions shown in reactions 3 and 4:
(2)
(3)
(4)
Therefore, in the presence of trace amounts of iron, the reaction of superoxide and hydrogen peroxide will form the destructive hydroxyl radical and initiate the oxidation of organic substrates. Metals other than iron may also participate in these electron transfer reactions by cycling between oxidised and reduced states.
The oxidation of organic substances may proceed by two possible reactions Ä addition of OH to the organic molecule, or abstraction of a hydrogen atom from it. In the addition reaction (reaction 5), the hydroxyl radical adds to an organic substrate forming a hydroxylated product that is further oxidised by ferrous ions, oxygen or other agents to a stable, oxidised product (reactions 6 and 7). The hydroxylated products can also dismutate to form cross-linked products (reaction 8).
(5)
(6)
(7)
(8)
In the abstraction reaction, the hydroxyl radical oxidises the organic substrate forming water and an organic radical (reaction 9). The latter product has a single unpaired electron and thus can react with oxygen in the triplet ground-state (reaction 10). The addition of triplet oxygen to the carbon radical can lead to the formation of a peroxyl radical which can readily abstract hydrogen from another organic molecule leading to the formation of a second carbon radical (reaction 11). This chain reaction is why oxygen free radicals cause damage far in excess of their initial concentration.
(9)
(10)
(11)
BIOLOGICAL REACTIONS OF OXYGEN RADICALS
The reactions of activated oxygen with organic substrates are complex even in vitro with homogenous solutions, but in biological systems there are even more complications due to the surface properties of membranes, electrical charges, binding properties of macromolecules, and compartmentalisation of enzymes, substrates and catalysts. Thus, various sites even within a single cell differ in the nature and extent of reactions with oxygen.
The nature of the oxidative injury that causes cell death is not always obvious. The mechanisms by which oxygen radicals damage membrane lipids are well accepted, and consequently oxidative damage is often exclusively associated with these peroxidation reactions in membrane lipids. What is sometimes overlooked in our research on environmental stress in plants is that activated forms of oxygen also degrade proteins and nucleic acids, reactions which can also be very lethal. In this section some of the major reactions of activated oxygen with lipids, protein, and nucleic acids are reviewed.
OXIDATIVE DAMAGE TO LIPIDS
Classical Peroxidation Reactions
The reactions of oxygen free radicals with polyunsaturated lipids have been extensively researched because of their involvement in rancidity and the development of undesirable odours and flavours in foods. Historically these reactions are the most frequently cited consequence of oxygen radical production in plant cells. Perhaps the mechanisms were so well established by oil chemists long before the recognition of their importance in biology that plant biologists applied these mechanisms directly to their experimental systems, rarely questioning their validity or transposability. This has delayed recognition of the presence of free radical reactions in plant membranes. The complexity of the biological membrane is well established and the reader is referred elsewhere for more detailed considerations of its structure (Leshem, 1992). The lipid bilayer membrane is composed of a mixture of phospholipids and glycolipids that have fatty acid chains attached to carbon 1 and 2 of the glycerol backbone by an ester linkage. The peroxidation reactions differ among these fatty acids depending on the number and position of the double bonds on the acyl chain and the reader is referred to Frankel (1985) for a detailed review. The following is a simplified summary of these reactions for a general lipid, `R’, and for a specific fatty acid, linoleate, which is common in plant cell membranes.
The peroxidation of lipids involves three distinct steps: initiation, propagation and termination. The initiation reaction between an unsaturated fatty acid (e.g. linoleate) and the hydroxyl radical involves the abstraction of an H atom from the methylvinyl group on the fatty acid (reaction 9); in the case of linoleate this occurs at carbon-11 (Fig. 3). The remaining carbon centred radical, forms a resonance structure sharing this unpaired electron among carbons 9 to 13. In the propagation reactions, this resonance structure reacts with triplet oxygen, which remember is a biradical having two unpaired electrons and therefore reacts readily with other radicals. This reaction forms a peroxy radical (reaction 10). In the case of linoleate, addition occurs at either carbon-9 or -13 (Fig 3). The peroxy radical then abstracts an H atom from a second fatty acid forming a lipid hydroperoxide and leaving another carbon centred free radical (reaction 11) that can participate in a second H abstraction (reaction 10). Therefore, once one hydroxyl radical initiates the peroxidation reaction by abstracting a single H atom, it creates a carbon radical product (R) that is capable of reacting with ground state oxygen in a chain reaction. The role of the hydroxyl radical is analogous to a “spark” that starts a fire. The basis for the hydroxyl radical’s extreme reactivity in lipid systems is that at very low concentrations it initiates a chain reaction involving triplet oxygen, the most abundant form of oxygen in the cell.
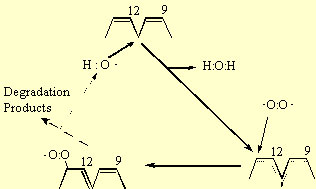
The lipid hydroperoxide (ROOH) is unstable in the presence of Fe or other metal catalysts because ROOH will participate in a Fenton reaction leading to the formation of reactive alkoxy radicals:
(12)
Therefore, in the presence of Fe, the chain reactions are not only propagated but amplified. Note that two radicals are produced by the summation of reactions 9 to 12. Among the degradation products of ROOH are aldehydes, such as malondialdehyde, and hydrocarbons, such as ethane and ethylene, that are commonly measured end products of lipid peroxidation.
The peroxidation reactions in membrane lipids are terminated when the carbon or peroxy radicals cross-link to form conjugated products that are not radicals, such as those shown in reactions 13 to 15:
(13)
(14)
(15)
Typically, high molecular weight, cross-linked fatty acids and phospholipids accumulate in peroxidised membrane lipid samples.
Singlet oxygen can react readily with unsaturated fatty acids producing a complex mixture of hydroperoxides. Again, the chemistry of these reactions is based on foods (Bradley and Minn, 1992). Oxidation of unsaturated fatty acids by singlet oxygen produces distinctly different products than the hydroxyl radical (Bradley and Minn, 1992). Once formed the lipid hydroperoxides will decompose into a variety of products, some of which can produce oxygen free radicals in the presence of metal catalysts (reaction 12).
Unique Reactions in Plant Membranes
The above mechanisms predict that oxygen free radical or lipid peroxidation reactions in plant membranes would selectively degrade unsaturated fatty acids and accumulate aldehydes, hydrocarbons, and cross-linked products. When examining the effects of environmental stresses on plant membranes, many studies have measured the products of lipid peroxidation, such as malondialdehyde and/or ethane and concluded that oxygen free radicals are involved in these stress responses. When the substrates of these reactions, the membrane fatty acids, have been examined, it has been very often observed that the unsaturated fatty acids are not selectively degraded, and therefore these reports have concluded that oxygen free radicals are not involved in these stress responses. This controversy has caused many to rule out the involvement of oxygen free radicals in processes such as seed ageing (Wilson and McDonald, 1986). However, in vitro experiments that have treated plant membranes with Fenton reaction products have shown that degradation of plant membrane lipids by oxygen free radicals does not involve selective loss of unsaturated fatty acids. For example, in Table 1, microsomal membranes isolated from wheat (Triticum aestivum) crowns and liposomes prepared from a commercial preparation of soybean asolecithin were treated in vitro with oxygen radicals generated by Fe-ascorbate. In both samples, there was destruction of fatty acids and their recovery from solution was lower after the free radical treatment. In the liposome sample there was selective degradation of unsaturated fatty acids; the proportion of linoleic and linolenic acids relative to the other fatty acids declined. In contrast, treatment of the wheat microsomal membranes caused degradation og the phospholipids but no change in the proportion of the fatty acids. Inother words, there was not selective degradation of the unstaurated fatty acids. Clearly other reactions to those described above were occurring in these plant membranes.
An alternative to the classical mechanism of lipid peroxidation was proposed by Niehaus (1978) based on his observation that most esters react with superoxide by cleaving the C-O bond. Since the fatty acid chains are attached to the glycerol backbone of the phospholipid molecule by an ester bond (Fig. 4), superoxide attack on a phospholipid bilayer would produce free fatty acids by de-esterification reactions. This was experimentally observed by Senaratna et al. (1985) in microsomal membranes from soybean seed axes treated in vitro with superoxide from xanthine oxidase.
Table 1: Degradation of phospholipid and esterified fatty acid in two membrane systems by Fenton reaction products. Data for each fatty acid are expressed as its proportion (%) of all fatty acids recovered (i.e.each sample totals 100%). Phospholipid (PL) recovery is expressed as a % of the original PL before treatment. Adapted from McKersie et al. (1990) | ||||
Fatty Acid | Liposomes | Wheat Microsomes | ||
Before | After | Before | After | |
16:0 | 21 | 34 | 29 | 30 |
18:0 | 3 | 5 | 1 | 1 |
18:1 | 7 | 10 | 6 | 7 |
18:2 | 61 | 47 | 28 | 29 |
18:3 | 8 | 4 | 37 | 35 |
PL Recovery | 100 | 51 | 100 | 65 |
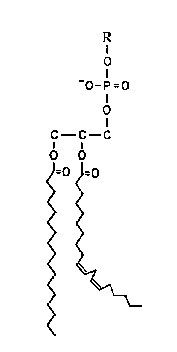
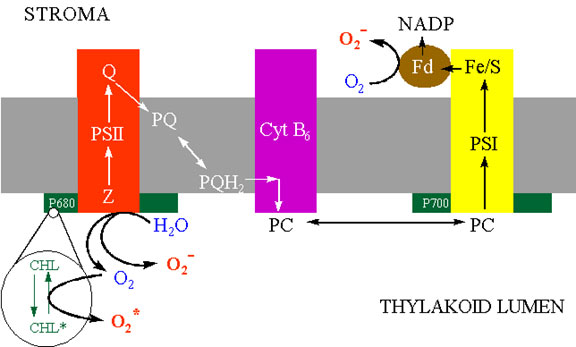
Kinetic analysis indicates that superoxide attack on esters occurs by a nucleophilic addition mechanism (Afanas’ev, 1985).
The peroxy radical RC(O)OO would abstract an H atom to form a hydroperoxide that would decompose into an acid RCOOÄ (Afanas’ev, 1985). In the case of a phospholipid, RCOOÄ would be a free fatty acid.
These reactions would not be selective for unsaturated fatty acids and therefore degradation of fatty acids attached to phospholipid molecules would be random. Experimentally if these reactions occurred in plant membranes, we would observe loss of phospholipids but no change in fatty acid unsaturation. Although this is commonly observed in stress conditions in plants, most researchers usually attribute these products to the action of an enzyme, such as phospholipase or a non-specific lipase.
Enzymatic and chemical reactions can clearly give the same products and the distinction between these mechanisms is clouded even further by the realisation that the formation of superoxide is the result of a dysfunctioning enzyme (see later section). Therefore, the debate is somewhat philosophical. Are there enzymes whose primary function is to degrade (turnover) phospholipid molecules during periods of stress? or are there enzymes that primarily function in redox transfer of electrons; but that dysfunction to form superoxide during stress?
It is not at all clear why some plant membranes such as the microsomal membranes from wheat crowns, exhibit de-esterification reactions instead of peroxidation reactions. Presumably, it is due to differences in composition, possibly the presence of specific lipid-soluble, membrane antioxidants (phenols, flavonoids, quinones), whose effect on free radical reactions are poorly understood.
OXIDATIVE DAMAGE TO PROTEINS
Oxidative attack on proteins results in site-specific amino acid modifications, fragmentation of the peptide chain, aggregation of cross-linked reaction products, altered electrical charge and increased susceptibility to proteolysis. The amino acids in a peptide differ in their susceptibility to attack, and the various forms of activated oxygen differ in their potential reactivity. Primary, secondary, and tertiary protein structures alter the relative susceptibility of certain amino acids. In spite of this complexity, generalisations can be made. Sulphur containing amino acids, and thiol groups specifically, are very susceptible sites. Activated oxygen can abstract an H atom from cysteine residues to form a thiyl radical that will cross-link to a second thiyl radical to form disulphide bridges. Alternatively, oxygen can add to a methionine residue to form methionine sulphoxide derivatives. Reduction of both of these may be accomplished in microbial systems by thioredoxin and thioredoxin reductase (Farr and Kogama, 1991). A protein-Methionine-S-oxide reductase has been measured in pea chloroplasts (Ferguson and Burke, 1992). This enzyme reduces the methionyl sulfoxide back to methionyl residues in the presence of thioredoxin (Brot and Weissbach, 1982). In some instances, this enzyme has restored the biological activity of a protein, but this function in plants has not been described.
Other forms of free radical attack on proteins are not reversible. For example, the oxidation of iron-sulphur centres by superoxide destroys enzymatic function (Gardner and Fridovich, 1991). Many amino acids undergo specific irreversible modifications when a protein is oxidised. For example, tryptophan is readily cross-linked to form bityrosine products (Davies, 1987). Histidine, lysine, proline, arginine, and serine form carbonyl groups on oxidation (Stadtman, 1986). The oxidative degradation of protein is enhanced in the presence of metal cofactors that are capable of redox cycling, such as Fe. In these cases, the metal binds to a divalent cation binding site on the protein. The metal then reacts with hydrogen peroxide in a Fenton reaction to form a hydroxyl radical that rapidly oxidises an amino acid residue at or near the cation binding site of the protein (Stadtman, 1986). This site-specific alteration of an amino acid usually inactivates the enzyme by destruction of the cation binding site.
Oxidative modification of specific amino acids is one mechanism of marking a protein for proteolysis (Stadtman, 1986). In E. coli there are specific proteases that degrade oxidised proteins (Farr and Kogoma, 1991) and similar specificity is expected in plants. It is well documented that the various peptide components of photosystem II turnover at different frequencies; the D1 protein specifically is noted for its high rate of turnover, and it is assumed that this is a consequence of oxidative attack at specific sites on the protein (Barber and Andersson, 1992).
OXIDATIVE DAMAGE TO DNA
Activated oxygen and agents that generate oxygen free radicals, such as ionising radiation, induce numerous lesions in DNA that cause deletions, mutations and other lethal genetic effects. Characterisation of this damage to DNA has indicated that both the sugar and the base moieties are susceptible to oxidation, causing base degradation, single strand breakage, and cross-linking to protein (Imlay and Linn, 1986). Degradation of the base will produce numerous products, including 8-hydroxyguanine, hydroxymethyl urea, urea, thymine glycol, thymine and adenine ring-opened and -saturated products.
The principle cause of single strand breaks is oxidation of the sugar moiety by the hydroxyl radical. In vitro, neither hydrogen peroxide alone nor superoxide cause strand breaks under physiological conditions, and therefore, their toxicity in vivo is most likely the result of Fenton reactions with a metal catalyst. At least in E. coli these Fenton reactions can be driven by NADH. For example, the ndh mutant in E. coli accumulates NADH as a result of the mutant’s inability to donate electrons from NADH to respiratory pathways; as a result, the mutant is hypersensitive to hydrogen peroxide. Studies of other E. coli mutants have led to the conclusion that a Fenton active metal is bound to DNA, probably chelated to phosphodiester linkage. If the bound metal is reduced by a small diffusible molecule, such as NAD(P)H or superoxide, it will react with hydrogen peroxide to form the hydroxyl radical (Imlay and Linn, 1986). The short-lived hydroxyl radical then oxidises an adjacent sugar or base causing breakage of the DNA chain.
Cross-linking of DNA to protein is another consequence of hydroxyl radical attack on either DNA or its associated proteins (Oleinick et al., 1986). Treatment with ionising radiation or other hydroxyl radical generating agents causes covalent leakages such as thymine-cysteine addicts, between DNA and protein. When these cross-linkages exist, separation of protein from DNA by various extraction methods is ineffective. Although DNA-protein cross-links are about an order of magnitude less abundant than single strand breaks, they are not as readily repaired, and may be lethal if replication or transcription precedes repair.
DNA is an obvious weak link in a cell’s ability to tolerate oxygen free radical attack. First, it seems that DNA is effective in binding metals that are involved in Fenton reactions, and secondly less damage can be tolerated in DNA than other macromolecules. As a consequence, the cell has a number of DNA repair enzymes (Beyer et al., 1991). One reason why eukaryotic organisms have compartmentalised DNA in the nucleus, away from sites of redox cycling that are high in NAD(P)H and other reductants, may be to avoid oxidative damage.
SITES OF ACTIVATED OXYGEN PRODUCTION
As indicated above, there are two forms of activated oxygen that are formed by distinctly different mechanisms. The reduction of oxygen to form superoxide, hydrogen peroxide and hydroxyl radicals is the principle mechanism of oxygen activation in most biological systems. However, in photosynthetic plants, the formation of singlet oxygen by the photosystems has importance. Activated oxygen is often formed as a component of metabolism to enable “complex” chemical reactions, such as the oxidation of xenobiotics or the polymerisation of lignin, but in other instances activated oxygen is formed by the dysfunctioning of enzymes or electron transport systems, as a result of perturbations in metabolism caused by chemical or environmental stress.
CHLOROPLASTS
As described by Elstner (1991), there are at least four sites within the chloroplast that can activate oxygen (Fig.6).
(1) PSI can reduce oxygen by the Mehler reaction which is an important mechanism of oxygen activation in the chloroplast. The reducing side of PSI is thought to contribute significantly to the monovalent reduction of oxygen under conditions where NADP is limiting. This would occur, for example, if the Calvin cycle did not oxidise NADPH as rapidly as PSI supplied electrons.
(2) Photoactivated chlorophyll normally transfers its excitation energy to the PS reaction centres, but under conditions that prevent the captured light energy from being utilised in the electron transport systems, this energy can excite oxygen from the triplet to singlet form. These conditions include stomatal closure caused by drought, damage to the membrane transport systems, lack of specific nutrients, or the presence of xenobiotic chemicals such as pollutants or herbicides.
(3) The oxidising side of PSII facilitates four single electron transfers from water to the PSII reaction centre releasing triplet or ground-state oxygen. Leaks of electrons from this site to molecular oxygen, or release of partially reduced oxygen products are thought to make a relatively minor contribution to activated oxygen production, but nonetheless it has been demonstrated that certain alcohols can be reduced by PSII.
- Photorespiration is the most obvious oxygenation pathway in the chloroplast. Rubisco catalyses the addition of oxygen to carbon 2 of RuBP forming phosphoglycolate and phosphoglycerate. Although this does not generate activated oxygen in the chloroplast, the subsequent metabolism of glycolate in the peroxisomes does.
MITOCHONDRIA
Most oxygen is consumed by the cytochrome oxidase enzyme in the mitochondrial electron transport system, and involves the sequential transfer of four electrons to oxygen, releasing water. Plant mitochondria have an additional site of oxygen reduction at the alternative oxidase, distinguished from cytochrome oxidase by its resistance to cyanide. However, neither of these sites produce significant quantities of superoxide (Rich and Bonner, 1978). However, isolated mitochondria produce H2O2 and O 2 in the presence of NADH (Loschen et al., 1973; 1974). Antimycin A, which blocks electron flow after ubiquinone (Fig. 7) enhances oxygen reduction. Presumably other conditions which also increase the reduction of ubiquinone favour reduction of oxygen in the ubiquinone Ä cytochrome b region of the chain (Rich and Bonner, 1978). The various Fe-S proteins and NADH dehydrogenase have also been implicated as possible sites of superoxide and hydrogen peroxide formation (Turrens et al., 1982).
ENDOPLASMIC RETICULUM
Various oxidative processes, including oxidation, hydroxylations, dealkylations, deaminations, dehalogenation and desaturation, occur on the smooth endoplasmic reticulum. Mixed function oxygenases that contain a heme moiety add an oxygen atom into an organic substrate using NAD(P)H as the electron donor. The generalised reaction catalysed by cytochrome P450 is:
(17)
The best characterised cytochrome P450 in plants is cinnamate-4-hydroxylase which functions in flavonoid and lignin biosynthesis, but other mixed function oxidases function in other biochemical pathways including gibberellin and sterol biosynthesis. Activation of oxygen by these systems is an essential prerequisite to oxygen addition reactions in the synthesis of these “complex” metabolites. Superoxide is produced by microsomal NAD(P)H dependent electron transport involving cytochrome P450 (Winston and Cederbaum, 1983). One possible site at which this may occur is shown in figure 7. After the univalent reduction of the substrate (RH) and the addition of triplet oxygen to form the complex P450 – RHOO the complex may decompose to P450-RH and release superoxide.
MICROBODIES
Peroxisomes and glyoxysomes are organelles with a single membrane that compartmentalises enzymes involved in the ß-oxidation of fatty acids, and the glyoxylic acid cycle including glycolate oxidase, catalase and various peroxidases. Glycolate oxidase produces H2O2 in a two electron transfer from glycolate to oxygen (Lindqvist et al., 1991). Xanthine oxidase, urate oxidase and NADH oxidase generate superoxide as a consequence of the oxidation of their substrates. The xanthine oxidase reaction is often used in vitro as a source of superoxide producing one mole of superoxide during the conversion of xanthine to uric acid (Fridovich, 1970).
PLASMA MEMBRANES
A superoxide-generating NAD(P)H oxidase activity has been clearly identified in plasmalemma enriched fractions (Vianello and Macri, 1991). These flavoproteins may produce superoxide by the redox cycling of certain quinones or nitrogenous compounds. In the root, NAD(P)H oxidase reduces Fe3+ to Fe2+ converting it to a form that can be transported. Dysfunction of this root enzyme will produce superoxide (Cakmak and Marschner, 1988). An auxin-activated NADH oxidase has been associated with acidification of the cell wall and auxin-stimulated cell elongation (Morré et al., 1988).
The plant NAD(P)H oxidase may have an analogous function to the animal enzyme. Leucocytes contain an NADH oxidase on the outer membrane surface which is activated in response to a foreign agent, generating superoxide that initiates oxidative reactions that destroy the potential pathogen (Hohn and Lehere, 1975). In plants, fungal elicitors cause a similar formation of superoxide that has been linked to the hypersensitive response to some pathogenic fungi (Doke and Ohashi, 1988; Doke et al., 1991). Wounding, heat shock and xenobiotics transiently activate this superoxide generating reaction, and consequently, it has been proposed that these superoxide generating reactions may serve as a signal in plant cells to elicit responses to biological, physical or chemical stress (Doke et al., 1991).
CELL WALLS
Although it is not immediately obvious, cell walls are active sites of metabolism, and also oxygen activation. Some of these reactions may be involved in the defense reactions against pathogens as described above. Others may involve the degradation or compartmentation of xenobiotic chemicals. However, the most common reactions are biosynthetic. For example, the phenylpropanoid precursors of lignin are crosslinked by H2O2 dependent reactions, that randomly link the subunits to form lignin (Gross, 1980). NADH is generated by a cell wall malate dehydrogenase, and then used to form H2O2 (Gross et al., 1977), possibly by the NADH oxidase on the plasmalemma (Vianello and Macri, 1991). Diamine oxidases are also involved in production of activated oxygen in the cell wall using diamines or polyamines (putrescine, spermidine, cadaverine, etc.) to reduce a quinone that will autoxidize, forming peroxides (Vianello and Macri, 1991; Elstner, 1991).
DEFENCE MECHANISMS
SUPEROXIDE DISMUTASE
Superoxide dismutase (SOD) was first isolated by Mann and Keilis (1938) and thought to be a copper storage protein. Subsequently, the enzyme was identified by a number of names, erythrocuprein, indophenol oxidase, and tetrazolium oxidase until its catalytic function was discovered by McCord and Fridovitch (1969). SOD is now known to catalyse the dismutation of superoxide to hydrogen peroxide and oxygen:
(18)
Therefore, the activity of this enzyme determines the relative proportions of the two constituents of the Haber-Weiss reaction that generates hydroxyl radicals (reaction 2). Since SOD is present in all aerobic organisms and most (if not all) subcellular compartments that generate activated oxygen, it has been assumed that SOD has a central role in the defence against oxidative stress (Beyer, et al., 1991; Bowler et al., 1992; Scandalias, 1993). There are three distinct types of SOD classified on the basis of the metal cofactor: the copper/zinc (Cu/Zn – SOD), the manganese (Mn-SOD) and the iron (Fe-SOD) isozymes (Bannister et al., 1987). These isozymes can be separated by native polyacrylamide gel electrophoresis, their activity detected by negative staining and identified on the basis of their sensitivity to KCN and H2O2. The Mn-SOD is resistant to both inhibitors, whereas the Cu/Zn-SOD is sensitive to both inhibitors; Fe-SOD is resistant to KCN, and sensitive to H2O2. The subcellular distribution of these isozymes is also distinctive. The Mn-SOD is found in the mitochondria of eukaryotic cells; some Cu/Zn-SOD isozymes are found in the cytosol, others in the chloroplasts of higher plants. The Fe-SOD isozymes are often not detected in plants, but when detected, Fe-SOD is usually associated with the chloroplast compartment (Bowler et al., 1992). The prokaryotic Mn-SOD and Fe-SOD, and the eukaryotic Cu/Zn-SOD enzymes are dimers, whereas the Mn-SOD of mitochondria are tetramers (Scandalias, 1993). Peroxisomes and glyoxysomes of watermelons (Citrillus vulgaris) have been shown to contain both Cu/Zn- and Mn-SOD activity (Sandalio and Del Rio, 1988), but there are no reports of extracellular SOD enzymes in plants. All forms of the SOD are nuclear-encoded and are targeted to their respective subcellular compartments by an amino terminal targeting sequence. Several forms of SOD have been cloned from a variety of plants (Scandalias, 1990; Bowler, 1992).
Prokaryotic cells, and many eukaryotic algae contain only the Mn-SOD and Fe-SOD isozymes which are believed to be more ancient forms. In the bacteria E. coli, SOD activity is transcriptionally regulated by the SOX RS operon (Farr and Kogoma, 1991) but investigations into the regulatory mechanism of SOD expression in plants are only beginning (Bowler et al., 1992). To date it has been shown that SOD activity is increased in cells in response to diverse environmental and xenobiotic stresses including paraquat, high light, waterlogging and drought. Apparently, each of the SOD isozymes are independently regulated according to the degree of oxidative stress experienced in the respective subcellular compartments, but how this is communicated at the molecular level is unknown. Bowler et al (1992) have suggested that this role may be served by unique lipid peroxidation products from each organelle that diffuse from the site of oxidative damage to the nucleus where they would enhance transcription of specific SOD genes.
Several reviews on superoxide dismutase have recently been published which describe the characteristics of the enzymes, the cloned cDNA sequences and genes, and the effects of overexpression in transgenic plants (Bowler, et al., 1994, Doke, et al., 1994, Foyer, et al., 1994, Gressel and Galun, 1994, Scandalios, 1993, Van Camp, et al., 1994)
CATALASE
Catalase is a heme-containing enzyme that catalyses the dismutation of hydrogen peroxide into water and oxygen. The enzyme is found in all aerobic eukaryotes and is important in the removal of hydrogen peroxide generated in peroxisomes (microbodies) by oxidases involved in ß-oxidation of fatty acids, the glyoxylate cycle (photorespiration) and purine catabolism. Catalase was one of the first enzymes to be isolated in a highly purified state. All forms of the enzyme are tetramers in excess of 220,000 molecular weight. Multiple forms of catalase have been described in many plants. These forms have been cloned from maize (Redinbaugh et al., 1988; Scandalias, 1990) and homologous genes has been cloned from several other plants. Maize has three isoforms termed cat-1, cat-2 and cat-3, that are on separate chromosomes and are differentially expressed and independently regulated (Scandalias, 1990). Cat-1 and cat-2 are localised in peroxisomes and the cytosol, whereas cat-3 is mitochondrial. Careful examination of the structure of beef liver catalase has shown four NADPH binding sites per catalase tetramer (Fita and Rossmann, 1985), but these sites were not in close association with the hydrogen peroxide reaction centre. Instead, NADPH functions in animal catalase to protect against inactivation by hydrogen peroxide (Kirkman et al., 1987). The only plant catalase examined, potato, does not contain NADPH (Beaumont et al., 1990). It is interesting in this regard to note that catalase is very sensitive to light and has a rapid turnover rate similar to that of the D1 protein of PSII (Hertwig et al., 1992). This may be a result of light absorption by the heme group or perhaps hydrogen peroxide inactivation. Regardless, stress conditions which reduce the rate of protein turnover, such as salinity, heat shock or cold, cause the depletion of catalase activity (Hertwig et al., 1992; Feirabend et al., 1992). This may have significance in the plant’s ability to tolerate the oxidative components of these environmental stresses.
ASCORBIC ACID
L-ascorbic acid (vitamin C) is an important vitamin in the human diet and is abundant in plant tissues. Green leaves have the same amount of ascorbate as chlorophyll. Because of its nutritional importance, the distribution of ascorbate has been extensively quantified in plants; however, relatively little consideration has been given to its function in the plant. Ascorbate has been shown to have an essential role in several physiological processes in plants, including growth, differentiation and metabolism (Foyer, 1993). Ascorbate functions as a reductant for many free radicals, thereby minimising the damage caused by oxidative stress but ascorbate may have other functions which remain undefined.
Apparently synthesis of ascorbate occurs in the cytosol because a specific ascorbate translocator has been identified on the chloroplast envelope. L-ascorbic acid is synthesised from hexose sugars in higher plants but controversy remains concerning some steps in its synthesis (Loewus, 1988). Although two distinct pathways are possible (Foyer, 1993), higher plants primarily convert D-glucose to ascorbate by a direct conversion that maintains the carbon chain in the same sequence. The pathway involves the oxidation of carbon-1 of D-glucose and enediol formation between carbons 2 and 3:
(19) D-glucose D-glucosone L-sorbosone L-ascorbic acid
Ascorbate can directly scavenge oxygen free radicals with and without enzyme catalysts and can indirectly scavenge them by recycling tocopherol to the reduced form. By reacting with activated oxygen more readily than any other aqueous component, ascorbate protects critical macromolecules from oxidative damage. The reaction with the hydroxyl radical is limited only by diffusion.
The reaction with superoxide may serve a physiologically similar role to SOD:
(20)
2 O 2 + 2H+ + ascorbate 2H2O2 + dehydroascorbate
The reaction with hydrogen peroxide is catalysed by ascorbate peroxidase (Asada, 1992):
(21)
H2O2 + 2 ascorbate 2H2O + 2 monodehydroascorbate
The indirect role of ascorbate as an antioxidant is to regenerate membrane-bound antioxidants, such as a-tocopherol, that scavenge peroxyl radicals and singlet oxygen, respectively:
(22)
tocopheroxyl radical + ascorbate -tocopherol + monodehydroascorbate
The above reactions indicate that there are two different products of ascorbate oxidation, monodehydroascorbate and dehydroascorbate, that represent one and two electron transfers, respectively (Fig. 9). The monodehydroascorbate can either spontaneously dismutate (reaction 23) or is reduced to ascorbate by NAD(P)H monodehydroascorbate reductase (reaction 24):
(23) 2 monodehydroascorbate ascorbate + dehydroascorbate
(24) monodehydroascorbate + NAD(P)H ascorbate + NAD(P)
The dehydroascorbate is unstable at pH greater than 6 decomposing into tartrate and oxalate. To prevent this, dehydroascorbate is rapidly reduced to ascorbate by dehydroascorbate reductase using reducing equivalents from glutathione (GSH):
(25)
2 GSH + dehydroascorbate GSSG + ascorbate
Ascorbate has been found in the chloroplast, cytosol, vacuole and extra-cellular compartments of the cell. About 20-40% of the ascorbate in the mesophyll leaf cell is in the chloroplast. The chloroplast contains all the enzymes to regenerate reduced ascorbate from its oxidised products. Foyer and Halliwell (1976) proposed that hydrogen peroxide was dissipated in the chloroplast by the coupling of ascorbate and glutathione redox cycling as shown in figure 10. Many of the details of this pathway and characterisation of the enzymes has been conducted by K. Asada in Japan. Consequently, this sequence of reactions is referred to as the Halliwell-Asada pathway. Illuminated chloroplasts produce superoxide and hydrogen peroxide from sites on the thylakoids, most commonly PSI. Superoxide is converted into hydrogen peroxide by either spontaneous dismutation or by the SOD enzyme. Hydrogen peroxide is scavenged by ascorbate and the enzyme ascorbate peroxidase (Asada, 1992). The monodehydroascorbate has two routes of regeneration, one via monodehydroascorbate reductase, the other via dehydroascorbate reductase and glutathione. The terminal electron donor is NADPH. This pathway serves two functions. One is the detoxification of hydrogen peroxide that might otherwise participate is Fenton reactions, and the second in the oxidation of NADPH. The latter function is an apparently energy-consuming, wasteful process analogous to photorespiration. It might at first seem more logical that the chloroplast contain catalase because it would allow the dissipation of hydrogen peroxide without “wasting” NADPH. However, it should be realised that conditions favouring electron transfer from PSI to oxygen generally cause a high redox potential, i.e. high NADPH/NADP ratio. By reducing this redox potential through the Halliwell-Asada pathway, the tendency of PSI to reduce oxygen is minimised.
Although ascorbate metabolism has been studied in most detail in the chloroplast, it is likely that all enzymes for its regeneration also exist in the cytosol of both photosynthetic and non-photosynthetic cells. For example, different isozymes for ascorbate peroxidase have been shown to exist in the cytosol and chloroplast compartments (Chen and Asada, 1989). The cell wall is also an important site of ascorbate metabolism because it contains mM concentrations of ascorbate. Here, ascorbate may have a role in cell wall biosynthesis (Polle et al., 1990). The cell wall does not contain ascorbate peroxidase, but contains ascorbate oxidase (Chichiricco et al., 1989). This enzyme contains 8-12 copper molecules per enzyme and catalyses the reaction:
(26)
2 ascorbates + O2 + 2H+ 2 dehydroascorbate + 2H2O
Since the enzymes to recycle oxidised forms of ascorbate are not present in the cell wall, it has been proposed that the plasmalemma may have an ascorbate translocator to shuttle oxidised and reduced forms between the cytosol and cell wall (Foyer, 1993).
GLUTATHIONE
Glutathione (GSH) is a tripeptide (Glu-Cys-Gly) whose antioxidant function is facilitated by the sulphydryl group of cysteine (Rennenberg, 1982). On oxidation, the sulphur forms a thiyl radical that reacts with a second oxidised glutathione forming a disulphide bond (GSSG). Some legumes contain homoglutathione (hGSH) that is a homologous tripeptide of Glu-Cys-Ala (Klapheck, 1988). Detailed reviews of GSH chemistry are available elsewhere (Alscher, 1989; Hausladen and Alscher, 1993). GSH has a redox potential of -340 mV that enables GSH to reduce dehydroascorbate to ascorbate or to reduce the disulphide bonds of proteins.
GSH is found in most tissues, cells and subcellular compartments of higher plants. Its levels declines with tissue age and vary among growth environments; for example, glutathione levels are higher in the light than in the dark. At the subcellular level, GSH concentration is highest in the chloroplast, averaging between 1 and 4 mM, but significant quantities also accumulate in the cytosol. GSH exists predominantly in the reduced form with estimates varying from 70% in barley chloroplasts (Smith et al., 1985) to 90% in pea chloroplasts (Bielawski and Joy, 1986).
GSH can function as an antioxidant in many ways. It can react chemically with singlet oxygen, superoxide and hydroxyl radicals and therefore function directly as a free radical scavenger. GSH may stabilise membrane structure by removing acyl peroxides formed by lipid peroxidation reactions (Price et al., 1990). As detailed in section 2.4.3, GSH is the reducing agent that recycles ascorbic acid from its oxidised to its reduced form by the enzyme dehydroascorbate reductase (Loewus, 1988). GSH can also reduce dehydroascorbate by a non-enzymatic mechanism at pH > 7 and GSH concentrations greater than 1 mM. This may be a significant pathway in chloroplasts whose stromal pH in the light is about 8 and GSH concentrations may be as high as 5 mM (Foyer and Halliwell, 1976).
There are alternative functions for GSH in cellular metabolism independent of its antioxidant properties. It may have a significant role in the transport of reduced sulphur from leaves to sink tissues such as the root (Rennenberg, 1982). GSH also participates in the detoxification of xenobiotics as a substrate for the enzyme Glutathione-S-transferase. The well documented tolerance of maize to the triazine herbicides is the result of conjugation of GSH to the herbicide (Timmerman, 1989). GSH is also the precursor of the phytochelatins that act as heavy metal binding peptides in plants (Rüegsegger et al., 1990).
The synthesis and degradation of GSH occurs continuously through the glutamyl cycle that has been well characterised in animals (Meister, 1988) and at least portions have been confirmed in plants (Hell and Bergman, 1988; 1990). The first step in GSH synthesis (reaction 27) is the combination of glutamate and cysteine to form glutamylcysteine by the enzyme glutamylcysteine synthetase. The subsequent step involves the addition of glycine by the enzyme glutathione synthetase (reaction 28). In the legumes that accumulate hGSH, this second step involves the addition of alanine by the enzyme homoglutathione synthetase (reaction 29).
(27) Glu + Cys Glu-Cys
(28) Glu-Cys + Gly Glu-Cys-Gly
(29) Glu-Cys + Ala Glu-Cys-Ala
The degradation of GSH involves first the cleavage of the bond between glutamate and cysteine by glutamyl transpeptidase and the transfer of the glutamate residues to an acceptor amino acid:
Subsequently the Cys-Gly dipeptide is degraded by dipeptidases and Glu-aa by glutamylcyclotransferase:
(30) Glu-Cys-Gly + aa Glu-aa + Cys-Gly
(31) Cys-Gly Cys + Gly
(32) Glu-aa 5-oxoproline + aa
Alternatively, plants may contain an additional pathway to that described in animals for degradation of GSH involving a GSH-specific carboxypeptidase and glutamylcyclotransferase (Steinkamp and Rennenberg, 1984):
(33) Glu-Cys-Gly Glu-Cys + Gly
(34) Glu-Cys 5-oxoproline + Cys
The presence of the alternative degradative pathway in plants is significant, because Glu-Cys is a common intermediate in the synthesis and degradation of GSH and thus may be a site for regulating GSH content in plant cells (Hausladen and Alscher, 1993). The final step in the degradation is the conversion of 5-oxoproline to glutamate by 5-oxoprolinase:
(35) 5-oxoproline Glu
The enzymes that catalyse the synthesis and degradation of GSH have been characterised in both the chloroplastic and cytosolic compartments (Hausladen and Alscher, 1993). The numerous inhibitors of these enzymes have been used in animal systems to characterise the function of GSH in cellular metabolism but their use in plant systems has not been extensive. Similarly, although a number of environmental stresses have been shown to cause GSH accumulation (Alscher, 1989), the mechanism involved has not been defined.
The reduction of GSSG to GSH is catalysed by the enzyme glutathione reductase (GR) which has been purified from a number of plant tissues (Smith et al., 1989), and cDNA for GR has been cloned from pea (Creisson et al., 1992). The amino acid sequences and structures as determined by x-ray crystallography are very similar among the human and E. coli GR so far examined, suggesting that this is a very highly conserved enzyme (Hausladen and Alscher, 1993). Nonetheless, the plant literature has conflicting reports of the subunit composition of GR; heterotetramer or homodimer forms have been reported (Hausladen and Alscher, 1993). This is not totally unexpected. Although animals have only one form of GR, plants have multiple forms of the enzyme. For example, pea leaf GR has been resolved into eight isoenzymes by two dimensional gel electrophoresis (Edwards et al., 1990). These isozymes are most likely associated with different subcellular compartments; GR is associated mainly with the chloroplast but significant activity is also found in the cytosol and a lesser amount in the mitochondria. The different isozymes quite probably represent the products of different genes with slightly or perhaps significantly different sequences, and with differing regulation and responses to environmental signals.
Animals contain a selenium containing enzyme, glutathione peroxidase, that reduces hydrogen peroxide forming GSSG and thereby serves as an alternative means of detoxifying activated oxygen. This enzyme was thought to be absent from higher plants (Smith and Shrift, 1979) but recently there have been reports of glutathione peroxidase in cultured cells ((Drotar et al., 1985; et al. 1991). A plant cDNA showing homology to animal glutathione peroxidase has been isolated from Nicotiana sylvestris (Criqui et al., 1992).
The tocopherols, specifically a-tocopherol (vitamin E), have been studied extensively in mammalian research as membrane stabilisers and multifaceted antioxidants, that scavenge oxygen free radicals, lipid peroxy radicals, and singlet oxygen (Diplock et al., 1989). This role is related to its fully substituted benzoquinone ring and fully reduced phytyl chain (Fig. 11). Its hydrophobic nature dictates that a-tocopherol is located exclusively in cell membranes and is oriented with the benzoquinone ring in close association with the carbonyl of the glycerol component of the phospholipid, and with the phytyl chain associated with the fatty acids in the hydrophobic inner regions of the membrane bilayer. The ring oxygen is near the surface of the membrane bilayer but remains exposed to the lipid environment.
Because of its dietary importance tocopherol levels have been documented extensively in plant tissue (Hess, 1993). Tocopherol concentrations vary among plant tissues from 200 ng g-1 fresh weight (fw) in potato tubers to 5 mg g-1 fw in oil palm leaflets. Tocopherol has been found in all higher plants, in both photosynthetic and non-photosynthetic tissues. Although tocopherol is a well-documented component of chloroplast membranes, there are no quantitative estimates of its distribution among other plant cell membranes.
Tocopherol is actually a family of antioxidants (Hess, 1993) that includes four tocols that have a phytyl chain and analogous tocotrienols that have a geranylgeranyl chain (Fig. 11j). a-Tocopherol is generally considered to be the most active form of the tocols; the other forms may be biosynthetic precursors. The relative synthesis of the tocols and tocotrienols may depend on the relative availability of phytyl and geranylgeranyl precursors. a-Tocopherol synthesis occurs in plastids with the aromatic ring formed by the shikimic acid pathway and the phytyl chain synthesized from geranylgeranyl pyrophosphate through the terpenoid pathway on the plastid envelope (Fryer, 1992). The latter forms a common link among the synthesis of chlorophyll, tocopherol, and carotenoids.
a-Tocopherol is well established as a membrane stabilising agent. Although some of this activity is due to its influence on membrane lipid organisation, at least part of this activity is the result of its ability to complex free fatty acids (Fryer, 1992). Free fatty acids act as detergents in membranes causing disruption of the bilayer, membrane aggregation and fusion. The association between the carboxyl group of the fatty acid and the ring of tocopherol reduces this destabilisation.
The antioxidant properties of tocopherol are the result of its ability to quench both singlet oxygen and peroxides (Fryer, 1992). Tocopherol is a less efficient scavenger of singlet oxygen than ßcarotene and therefore in the thylakoid membrane it may function to break carbon radical chain reactions by trapping peroxyl radicals:
(36) ROO + tocopherol ROOH + tocopherol
The tocopheroxyl radical is stabilised by the fully substituted benzoquinone ring and therefore does not propagate the radical reaction. This is in effect a termination reaction making tocopherol an effective free radical trap.
Because the active oxygen of the a-tocopherol is located near the surface of the bilayer and because it readily diffuses laterally in the plane of the bilayer, tocopherol can react with peroxyl radicals formed in the bilayer as they diffuse to the aqueous phase. This position also allows the tocopheroxyl radical to be reduced by ascorbate in the aqueous phase to regenerate a-tocopherol (reaction 22). Glutathione reduces tocopheroxyl radicals in alcohol solutions, but there is only limited evidence for this in biological systems (Hess, 1993).
CAROTENOIDS
Carotenoids are C40 isoprenoids and tetraterpenes that are located in the plastids of both photosynthetic and non-photosynthetic plant tissues. In chloroplasts, the carotenoids function act as accessory pigments in light harvesting, but perhaps a more important role is their ability to detoxify various forms of activated oxygen and triplet chlorophyll that are produced as a result of excitation of the photosynthetic complexes by light.
There are two classes of carotenoids. The carotenes are hydrocarbons; the xanthophylls are carotene derivatives that contain one or more oxygen atoms. Carotenoids are synthesised from geranylpyrophosphate from the isoprenoid pathway in plastids, and thus have common precursors to chlorophyll and tocopherol. ß-carotene is formed by the cyclisation of lycopene and the xanthophylls are formed by mixed function oxidases that introduce hydroxyl groups to the carotene molecule. Details of these biosynthetic pathways have been recently reviewed (Young, 1991a; Pallett and Young, 1993). Certain classes of herbicides function by the inhibition of carotenoid biosynthesis (Young, 1991a).
The carotenoids can exist in a ground state or in one of two excited states after the absorption of light energy. Details of carotenoid photochemistry are given elsewhere (Young, 1991b). In terms of its antioxidant properties carotenoids can protect the photosystems in one of four ways: by reacting with lipid peroxidation products to terminate chain reactions (Burton and Ingold, 1984); by scavenging singlet oxygen and dissipating the energy as heat (Mathis and Kleo, 1973); by reacting with triplet or excited chlorophyll molecules to prevent formation of singlet oxygen; or by the dissipation of excess excitation energy through the xanthophyll cycle.
Carotenoids may augment a-tocopherol in scavenging peroxy radicals (Burton and Ingold, 1984):
(37) ß-car + ROO ß-car + ROOH
(38) ß-car + ROO inactive products
These reactions therefore act as chain terminations but unlike tocopherol a mechanism to recycle ß-carotene to the reduced form has not been described.
The main protective role of ß-carotene in photosynthetic tissue may be through its direct quenching of triplet chlorophyll, which would prevent the generation of singlet oxygen, and therefore completely avoid oxidative stress.
(39) 3Chl* + 1ß-car 1Chl + 3ß-car*
(40) 3ß-car* 1ß-car + heat
In reactions 39 and 40, energy is transferred from chlorophyll to a carotenoid which subsequently dissipates the energy in a non-radiative form (heat). Thus, the carotenoids act as a competitive inhibitor for the formation of singlet oxygen, and this is aided considerably by their proximity to chlorophyll in the light harvesting complex. This method of protection is especially critical as light intensity increases above saturating levels (Demming-Adams and Adams, 1993). Another carotenoid, zeaxanthin, has been implicated in the dissipation of thermal energy, but the precise mechanism has not been resolved. Zeaxanthin apparently facilitates the conversion of triplet to singlet chlorophyll in more efficient manner than ß-carotene. The xanthophyll cycle involves the reversible conversion of the xanthophylls between two forms, violaxanthin and zeaxanthin. A de-epoxidase enzyme catalyses the de-epoxidation of violaxanthin to zeaxanthin in the presence of excess light, and an epoxidase catalyses the reverse reaction in darkness or low light. Zeaxanthin therefore accumulates under light intensities that exceed photosynthetic capacity. The de-epoxidase has a low pH optimum (5.1), whereas the epoxidase has a high pH optimum (7.5). Reduced ascorbate serves as the electron donor for the de-epoxidase, whereas NADPH supplies reducing equivalents for the epoxidase (Fig. 13).
HERBICIDE TOLERANCE
A number of herbicides that are widely used on many agricultural crops act by the production of oxygen free radicals. A few plants have evolved or have been selected for tolerance to these herbicides. The mechanism of tolerance in some plants is related to the plants’ ability to scavenge activated oxygen and thereby avoid oxidative stress. Two examples will be discussed in detail.
PARAQUAT
Paraquat and diquat are bipyridylium (viologen) herbicides that are effective as non-selective herbicides when applied to leaves. Symptoms include rapid wilting and desiccation of the leaf, followed by necrosis with 24 hours. Both light and chlorophyll are required for rapid response to the herbicide, which suggested to the early researchers that the herbicide was acting on photosynthesis. In the dark and in etiolated seedlings the symptoms appear more slowly (Mees, 1960). At the ultrastructural level, the first injury symptoms are chloroplast swelling, followed by breakdown of the tonoplast and the plasmalemma, and then breakdown of the thylakoids and chloroplast envelope (Dodge and Lawes, 1974). These observations indicated that cellular organelles differ in their sensitivity to the toxic agent and that the toxic agent is able to diffuse within the cell from its site of production in the chloroplast to the tonoplast and plasmalemma where it has its action promotes the visible symptoms of wilting and necrosis.
That the toxic agent is some form of activated oxygen was suggested by the first experiments of Mees (1960) who postulated that hydrogen peroxide was produced from the photosynthetic electron transport system. This was based on the observations that monuron, an inhibitor of the Hill reaction, slowed the phytotoxic symptoms. Calderbank (1968) proposed that the hydroxyl radical was produced by these reactions, and subsequently Farrington et al. (1973) suggested the involvement of superoxide.
Paraquat has a redox potential of -446 mV, which is critical to its function as a free radical generator. Any reducing agent with sufficient energy can denote an electron to the bipyridylium divalent cation, paraquat2+, to form a free radical, paraquat1+. The oxidation of the bipyridylium radical to form the original paraquat2+ results in the transfer of the electron to oxygen and the formation of superoxide (Fig. 14). Subsequent Haber-Weiss and Fenton reactions yield toxic hydroxyl radicals. The paraquat2+ ion is again ready for another cycle, and thus the herbicide functions as a catalyst to transfer reducing equivalents to oxygen. In the leaf, the major reducing agent with sufficient energy to reduce paraquat is the primary electron acceptor in PSI, ferredoxin, but other reducing agents may be effective. Consequently, bipyridylium herbicides are also quite toxic to non-photosynthetic organisms, including humans.
Resistance to paraquat has appeared in a number of weedy species after prolonged use of the herbicide (Fuerst and Vaughn, 1990), in Lolium perenne after breeding and selection (Harper and Harvey, 1978), and in genetically engineered plants, animal cells, and microbes (see below). In paraquat-sensitive plants that have been selected in the field by prolonged exposure paraquat, the mechanism(s) of resistance is ambiguous. In biotypes of Conyza baraciensis (Shaaltiel and Gressel, 1986) and in perennial ryegrass, Lolium perenne (Harper and Harvey, 1978), resistance was correlated with increased activity of superoxide dismutase and other free radical scavenging enzymes. However, in other resistant plants there is reduced translocation of the herbicide to its site of action in the chloroplast (Fuerst et al., 1985; Preston et al., 1992), and in other instances, a correlation with superoxide dismutase activity was not observed (Vaughn and Fuerst, 1985).
Unfortunately, the situation is just as ambiguous in genetically engineered organisms that have modified expression of superoxide dismutase or glutathione reductase. Gruber et al. (1990) expressed the E. coli Mn-superoxide dismutase in the cyanobacterium Anacystis nidulans, and observed that the transformants had less damage when treated with paraquat. Similarly, Bowler et al. (1991) expressed a Mn-superoxide dismutase cDNA from Nicotiana plumbiginfolia in N. tabacum and showed reduced cellular damage when the transformed plants were treated with paraquat. However, tomato and tobacco plants that were transformed with a Cu/Zn-superoxide dismutase cDNA from Petunia did not have enhanced paraquat resistance (Tepperman and Dunsmuir, 1990).
Superoxide dismutase is unlikely to be the only enzyme involved in providing protection against activated oxygen produced by paraquat. Glutathione peroxidase has been associated with paraquat resistance in a human cell line, HL60 (Kelner and Bagnell, 1990). A transgenic N. tabacum plant that expresses the glutathione reductase gene from E. coli had reduced susceptibility to paraquat compared to the control plants based on visual leaf damage (Aono et al., 1991). Malan et al. (1990) observed that in maize inbreds that tolerance of paraquat (and also drought) was not significantly correlated with the activity of either superoxide dismutase or glutathione reductase individually, but it was correlated with the two enzyme activities collectively. Glutathione reductase reduces production of reduced oxygen by isolated chloroplasts in the presence of paraquat presumably by restoration of the NADP pool (Härtl et al., 1992).
There are also developmental changes in the plant’s tolerance to paraquat. For example, in winter wheat, mesophyll protoplasts, excised leaves, and seedlings have increased tolerance to paraquat after acclimation of the seedlings at 2°C (Kendall and McKersie, 1989; Bridger et al., 1994). This was associated with an increased quantity of lipid soluble antioxidants that would minimise the deleterious chain reactions associated with lipid peroxidation (Kendall and McKersie, 1989).
The above discussion illustrates a generalisation which will be a common theme in the chapters to follow. Most often our experimental research involves simple experiments that have focused on individual components of stress tolerance, in this case herbicide tolerance. The plant is not so simple and as a general rule will not develop a single method of surviving a potentially catastrophic stress, if two or more can be developed in parallel. In some instances, discussed later these mechanisms of tolerance may be mutually exclusive, but very often they are complimentary and serve as backup systems, insurance or additive protection. Looking at the case in point, tolerance of the free radical generating herbicide paraquat, it is obvious that a number of mechanisms may be involved: (a) reduced translocation of the herbicide to its site of action; (b) altered redox potential of PSI primary electron acceptor so that the paraquat is not an efficient electron acceptor and will therefore not generate superoxide; (c) enhanced scavenging of superoxide produced by PSI; and (d) enhanced protection of membrane components from lipid peroxidation reactions. All the above mechanisms except (b) have been described in tolerant plants. Presumably mechanism (b) is not viable because changes in redox potential would also change the efficiency of electron transfer between ferredoxin and NADP, and this would reduce photosynthetic efficiency and competitiveness or yield. The actual mechanism(s) selected will undoubtedly vary among plants.
PHOTOSENSITISING HERBICIDES
Several herbicide formulations promote the accumulation of metabolic intermediates of chlorophyll, namely tetrapyrroles. In the light, photons are absorbed by the tetrapyroles and the energy used to create singlet oxygen that kills the plant. Two classes of photobleaching herbicides, the p-nitrodiphenyl ethers and aminolevulinic acid-based modulator, differ in the precise mechanisms of tetrapyrrole accumulation.
The p-nitrodiphenyl ethers, exemplified by acifluorfen, are used as selective herbicides in soybeans (Johnson et al., 1978) and require light for herbicidal activity. Early symptoms of injury include wilting, desiccation, and necrosis. Ultrastructural studies have shown that the plasmalemma and tonoplast are the first organelles to be disrupted, not the chloroplast, which explains why enhanced electrolyte leakage is one of the first indicators of injury from a photosensitising herbicide. Acifluorfen selectively inhibits protoporphyrinogen oxidase (Protox) which is the penultimate step in heme and Mg-protoporphyrin IX synthesis on the chloroplast envelope (Fig. 15) (Matrinage et al., 1989; Witkowski and Halling, 1989). This blockage results in the accumulation of its substrate protoporphyrinogen which at the time was thought to oxidise to its product protoporphyrin IX by a “spontaneous” reaction (Witkowski and Halling, 1989). The loss of enzymatic control of protoporphyrin IX synthesis was associated with lower heme levels and increased carbon flow into the pathway (Kouji et al., 1989). Because heme is a feedback inhibitor of aminolevulinic acid synthesis, this change in regulation of the pathway was thought to lead to the 100-fold accumulation of protoporphyrin IX (Matsumoto and Duke, 1990) but a detailed evaluation of the data from these early experiments on acifluorfen action were inconsistent with the herbicide acting solely via tetrapyrrole accumulation and other mechanisms have been proposed (Mayasick et al., 1990; Rebeiz et al., 1990). Subsequently, in vivo localisation studies have shown that tetrapyrroles accumulate at sites outside of the chloroplast such as the plasmalemma, which may explain why photosensitising damage is not seen initially in the chloroplast (Lehnen et al., 1990).
The presumed “spontaneous” oxidation of protoporphyrinogen IX to protoporphyrin IX is apparently accomplished by a protoporphyrinogen oxidising enzyme outside of the chloroplast, probably on the plasmalemma (Jacobs et al., 1991). This enzyme is presumably less sensitive than the chloroplast enzyme to the nitrodiphenyl ether inhibitors, and the presence of this second enzyme would explain many of the inconsistencies of the earlier data (Robeiz et al., 1990), the observed accumulation of protoporphyrin IX outside of the chloroplast, and the observed depletion of heme in the chloroplast. The major mechanism of action of acifluorfen and other diphenyl either herbicides is now thought to involve a blockage of the chloroplastic pathway shunting the protoporphyrinogen to an extra-chloroplastic pathway that first uncouples the regulation of the tetrapyrrole pathway on the chloroplast envelope by low heme levels, and secondly causes the accumulation of a photosensitising agent, protoporphrin IX in the cellular membranes.
The mode of action of the aminolevulinic acid-based herbicides is more clearly established because unlike other herbicides they were designed based on a knowledge of chlorophyll synthesis and free radical chemistry (Rebeiz et al., 1990). All formulations are based on the concept of supplying aminolevulinic acid to the plant as a precursor of tetrapyrrole synthesis (Fig. 15), in conjunction with a modulator that qualitatively and quantitatively alters the pattern of tetrapyrrole accumulation by selectively stimulating or inhibiting various steps in the biosynthetic pathway. The accumulated tetrapyrroles serve as photosensitizers that produce singlet oxygen in the light. The herbicide formulations are most effective if the sprayed plants remain in darkness for a few hours to allow tetrapyrrole accumulation before exposure to the light. After about 20 min illumination, the leaves develop isolated bleached areas that expand followed by wilting, desiccation and necrosis.
Although the photosensitising herbicides were assumed to be non-selective, laboratory and field trials have indicated that significant selectivity can be achieved by modifications of the formulations. This is caused by the different capacities of tissues and plants of accumulate tetrapyrroles and presumably to sequester or translocate the herbicides. Details of the mechanism of selectivity are given elsewhere (Rebeiz et al., 1990).
Some plants have apparently achieved increased resistance to acifluorfen by an enhanced scavenging capacity of oxygen free radicals (Gullner et al., 1991). Bean leaves treated with acifluorfen responded by higher levels of reduced glutathione and higher activity of glutathione reductase (Schmidt and Kunert, 1986). A tobacco line that was resistant to paraquat was also co-tolerant of acifluorfen (Gullner et al., 1991). This tolerance was associated with a greater inducibility of several components of the oxygen free radical scavenging system. Winter wheat (Triticum aestivum) seedlings that develop tolerance of freezing and of paraquat as they acclimate at 2°C, also develop increased resistance to acifluorfen (Bridger et al., 1994). Alfalfa plants that express a transgene of the Mn-SOD cDNA from Nicotiana have an increased LD50 for acifluorfen (McKersie et al., 1993).
SUMMARY AND CONCLUSIONS
Oxygen free radicals or activated oxygen has been implicated in diverse environmental stresses in plants and animals and appears to be a common participation in most, if not all, degenerative conditions in eukaryotic cells. The peroxidation of lipids, the cross-linking and inactivation of proteins and mutations in DNA are typical consequences of free radicals, but because the reactions occur quickly and often are components of complex chain reactions, we usually can only detect their “footprints”. Activated forms of oxygen are important in the biosynthesis of “complex” organic molecules, in the polymerisation of cell wall constituents, in the detoxification of xenobiotic chemicals and in the defence against pathogens. Thus, the plant’s dilemma is not how to eliminate the activation of oxygen, but how to control and manage the potential reactions of activated oxygen. Complex systems of scavenging activated oxygen therefore exist in plant cells with complimentary and interdependent strategies. Some components such as the carotenoids prevent the formation of activated oxygen by competing for the energy leaked from the photosystems. Other components are lipid soluble and reside in the membrane bilayer to terminate the lipid peroxidation chain reactions. Still others, ascorbate and glutathione, are aqueous scavenger that detoxify activated oxygen directly or serve to recycle other protective components back to their reduced state. The enzymes that catalyse the synthesis, degradation and recycling of these antioxidants are essential to viability. Consequently, they are highly conserved among plants, and exist in multiple forms in different subcellular compartments and different tissues to allow precise regulation.
There are numerous sites of oxygen activation in the plant cell, which are highly controlled and tightly coupled to prevent release of intermediate products. Under stress situations, it is likely that this control or coupling breaks down and the process “dysfunctions” leaking activated oxygen. This is probably a common occurrence in plants especially when we consider that a plant has minimal mobility and control of its environment. These uncoupling events are not detrimental provided that they are short in duration and that the oxygen scavenging systems are able to detoxify the various forms of activated oxygen. If the production of activated oxygen exceeds the plant’s capacity to detoxify it, deleterious degenerative reactions occur, the typical symptoms being loss of osmotic responsiveness, wilting, and necrosis. At the subcellular level, disintegration of membranes and aggregation of proteins are typical symptoms. Therefore it is the balance between the production and the scavenging of activated oxygen that is critical to the maintenance of active growth and metabolism of the plant and overall environmental stress tolerance.
REFERENCES
Afanas’ev, I.B. 1985. Superoxide Ion: Chemistry and Biological Implications Volume 1. CRC Press, Boca Raton.
Alscher, R.G. 1989. Biosynthesis and antioxidant function of glutathione in plants. Physiol. Plant. 77:457-464.
Aono, M., Kubo, A., Saji, H., Natori, T., Tanaka, K. and Kondo, N. 1991. Resistance to active oxygen toxicity of transgenic Nicotiana tabacum that expresses the gene for glutathione reductase from Escherichia coli. Plant Cell Physiol. 32:691-697.
Asada, K. 1992. Ascorbate peroxidase – hydrogen peroxide-scavenging enzyme in plants. Physiol. Plant. 85: 235-241.
Bannister, J.V., Bannister, W.H. and Rotils, G. 1987. Aspects of the structure, function and applications of superoxide dismutase. CRC Crit. Rev. Biochem. 22:110-180.
Barber, J. and Andersson, B. 1992. Too much of a good thing: light can be bad for photosynthesis. Trends Biochem. Sci. 17:61-66.
Beaumont, F., Jouvc, H-M., Cagnan, J., Gillard, J. and Pelment, J. 1990. Purification and properties of a catalase from potato tubers (Solanum tuberosum). Plant Sci. 72:19-26.
Beyer, W., Imlay, J., Fridovich, I. 1991. Superoxide Dismutases. Prog. Nucl. Acid Res. 40:221-253.
Bielawski, W. and Joy, K.W. 1986. Reduced and oxidized glutathione and glutathione-reductase activity in tissues of Pisum sativum. Planta, 169:267-272.
Bowler, C., Slooten, L., Vandenbranden, S., De Rycke, R., Botterman, J., Sybesma, C., Van Montagu, M. and Inzé, D. 1991. Manganese superoxide dismutase can reduce cellular damage mediated by oxygen radicals in transgenic plants. EMBO. J. 10:1723-1732.
Bowler, C. and Van Montague, M. and Inzé, D. 1992. Superoxide dismutase and stress tolerance. Ann Rev. Plant Physiol. Plant Mol. Biol. 43:83-116.
Bowler C., Van Camp W. , Van Montagu M. and Inze D. 1994. Superoxide dismutase in plants. Critical Rev. Plant Sci. 13: 199-218
Bradley, D.E., Min. D.B. 1992. Singlet oxygen oxidation of foods. Cat. Rev. Food Sci. Nutri. 31: 211-236.
Bridger, G.M., Yang, W., Falk, D.E. and McKersie, B.D. 1994. Cold acclimation increases tolerance of activated oxygen in winter cereals. J. Plant Physiol. 144:235-240.
Brot, N. and Weissbach, H. 1982. The biochemistry of methionine sulfoxide residues in proteins. Trends Biochem. Sci. 7:137-139.
Burton, G.W. and Ingold, K.U. 1984. ß-carotene: an unusual type of lipid antioxidant. Science. 224:569-573.
Cakmak, I. and Marschner, H. 1988. Enhanced superoxide radical production in roots of zinc-deficient plants. J. Expt. Bot. 39:1449-1460.
Calderbank, A. 1968. The bipyridylium herbicides. Adv. in Pest Control Res. 8:127-135.
Chen, G.X. and Asada, K. 1989. Ascorbate peroxidase in tea leaves – occurrence of 2 isozymes and the differences in their enzymatic and molecular properties. Plant and Cell Physiology. 30:987-998.
Chichiricco, G., Ceru, M.P., D’Alessandro, A., Oratore, A., and Avigliano, L. 1989. Immunohistochemical localisation of ascorbate oxidase in Cucurbita pepo medullosa, Plant Sci. 64:61-66.
Creisson, G., Edwards, E.A., Anard, C. Wellburn A. and Mullineaux, P. 1992. Molecular characterization of glutathione reductase cDNAs from pea (Pisum sativum L. ). Plant J. 2:129-131.
Criqui, M.C., Jamet, E., Parmentier, Y., Marbach, J., Darr, A. and Fleck, J. 1992. Isolation and characterization of a plant cDNA showing homology to animal glutathione peroxidases. Plant Mol. Biol. 18: 623-627.
Davies, K.J.A. 1987. Protein damage and degradation by oxygen radicals. I General aspects. J. Biol. Chem. 162:9895-9901.
Demmig-Adams, B. and Adams, W.W. 1993. The Xanthophyll Cycle. In: R.G. Alscher and J.L. Hess eds. Antioxidants in Higher Plants. CRC Press, Baco Raton pp. 59-90.
Diplock, A.T., Machlin, L.J., Packer, L., and Pryor, W.A. 1989. Eds., Vitamin E: Biochemistry and Health Implications. Ann. N.Y. Acad. Sci. Vol. 570 p.555.
Dodge, J.D. and Lawes, G.B. 1974. Some effects of the herbicides diquat and morfamquat on the fine structure of leaf cells. Weed Res. 14:45-49.
Doke, N. and Ohashi, Y. 1988. Involvement of an O2-generating system in the induction of necrotic lesions on tobacco leaves infected with tobacco mosaic virus. Physiol. Mol. Plant. Pathol. 32:163-175.
Doke, N., Miura, Y., Chai, H-B, Kawakita, K. 1991. Involvement of Active Oxygen in induction of plant defense response against infection and injury. In: Active Oxygen/Oxidative Stress and Plant Metabolism. Pell E.J. and Steffen K.L. (eds) American Soc. Plant Physiol. Rockville, M.D. pp. 84-96.
Doke N., Miura Y., Sanchez L. M. and Kawakita K. 1994. Involvement of superoxide in signal transduction: responses to attack by pathogens, physical and chemical shocks, and UV irradiation. In: C. H. Foyer and P. M. Mullineaux (eds). Causes of photooxidative stress and amelioration of defense systems in plants. CRC Press Inc. Boca Raton, FL, USA. pp. 177-197
Drotar, A., Phelps, P. and Fall, R. 1985. Evidence for glutathione peroxidase activities in cultured plant cells. Plant Science 42:35-40.
Edwards, E.A., Rawsthorne, S. and Mullineaux, P.M. 1990. Subcellular distribution of multiple forms of glutathione reductase in leaves of pea (Pisum sativum L.). Planta 180:278-284.
Elstner, E.F. 1982. Oxygen activation and oxygen toxicity. Ann. Rev. Plant Physiol. 33:73-96.
Elstner, E.F. 1991. Mechanisms of oxygen activation in different compartments of plant cells In: Active oxygen/oxidative stress and plant metabolism. Pell E.J. and Steffen K.L. (eds) American Soc. Plant Physiol. Rockville, M.D. pp. 13-25.
Farr, S.B. and Kogoma, T. 1991. Oxidative stress responses in Escherichia coli and Salmonella typhimurium. Microbiol. Rev. 55:561-585.
Farrington, J.A., Ebert, H., Land, E.J. and Fletcher, K. 1973. Bipyridylium quaternary salts and related compounds. V. Pulse radiolysis studies of the reaction of paraquat radical with oxygen. Implications for mode of action of bipyridyl herbicides. Biochim. Biophys. Acta 314:372-381.
Feierabend, J., Schaan, C., and Hertwig, B. 1992. Photoinactivation of catalase occurs under both high- and low-temperature stress conditions and accompanies photoinhibition of photosystem II. Plant Physiol. 100:1554-1561.
Fenton, H.J.H. 1894. Oxidation of tartaric acid in the presence of iron. J. Chem. Soc. 65:899.
Fenton, H.J.H. 1899. Oxidation of certain organic acids in the presence of ferrous salts. Proc. Chem. Soc. 25:224.
Ferguson, D.L. and Burke, J.J. 1992. A new method of measuring protein-methionine-S-oxide reductase activity. Plant Physiol. 100:529-532.
Fita, I. and Rossmann, M.G. 1985. The active center of catalase. J. Mol. Biol. 185:21-37.
Foyer, C.H. and Halliwell, B. 1976. The presence of glutathione and glutathione reductase in chloroplasts: a proposed role in ascorbic acid metabolism. Planta. 133:21-25.
Foyer, C., 1993. Ascorbic acid. In: Antioxidants in Higher Plants. R.G. Alscher and J.L. Hess (eds) CRC Press, Boca Raton, pp.31-58.
Foyer, C. H., Descourvieres P. and Kunert K. J. 1994. Protection against oxygen radicals: an important defence mechanism studied in transgenic plants. Plant, Cell and Environment 17: 507-523
Frankel, E.N. 1985. Chemistry of free radical and singlet oxidation of lipids. Progress in Lipid Research. 23:197-221.
Fridovich, I. 1970. Quantitative aspects of the production of superoxide anion radical by milk xanthine oxidase. J. Biol Chem 245:4053-4057.
Fryer, M.J. 1992. The antioxidant effects of thylakoid vitamin E (a-tocopherol). Plant Cell Environ. 15:381-392.
Fuerst, E.P., Nakatani, H.Y., Dodge, A.D., Penner, D. and Arntzen, C.J. 1985. Paraquat resistance in Conyza. Plant Physiol. 77:984-989.
Fuerst, E.P. and Vaughn, K.C. 1990. Mechanisms of paraquat resistance. Weed Technol. 4:150-156.
Gardner, P.R. and Fridovich, I. 1991. Superoxide sensitivity of Escherichia coli 6-phosphogluconate dehydratose. J. Biol. Chem. 266:1478-1483.
Gebicki, J.M. and Bielski, B.H.J. 1981. Comparison of the capacities of the perhydroxyl and superoxide radicals to initiate chain oxidation of linoleic acid. J. Am. Chem. Soc. 103: 7020-7022.
Gressel, J. and Galun E. 1994. Genetic controls of photooxidant tolerance. In: C. H. Foyer and P. M. Mullineaux (eds). Causes of photooxidative stress and amelioration of defense systems in plants. CRC Press Inc. Boca Raton, FL, USA. pp. 237-273.
Gross, G.G., Janse, C. and Elstner, E.F. 1977. Involvement of malate, monophenols and superoxide radical in hydrogen peroxide formation by isolated cell walls from horseradish. (Armoracia lapathifolia Gilib.) Planta 136:271-276.
Gross, G.G. 1980. The biochemistry of lignification. Adv. Bot. Res. 8:25-63.
Gruber, M.Y., Glick, B.R. and Thompson, J.E. 1990. Cloned manganese superoxide dismutase reduces oxidative stress in Escherichia coli and Anacystis nidulans. Proc. Nat. Acad Sci. (U.S.A.) 87:2603-2612.
Gullner, G., Komives, T. and Kiroly, L. 1991. Enhanced inducibility of antioxidant systems in a Nicotiana tabacum L. biotype results in acifluorfen resistance. Naturforsch. 46c:875-881.
Haber, F. and Weiss, J. 1934. The catalytic decomposition of hydrogen peroxide by iron salts. Proc. Royal Soc. A. 147:332.
Harper, D.B., Harvey, B.M.R. 1978. Mechanism of paraquat tolerance in perennial ryegrass. II. Role of superoxide dismutase, catalase and peroxidase. Plant Cell Environ. 1:211-215.
Härtel, H., Haseloff, R.F., Ebert, B., Rank, B. 1992. Free radical formation in chloroplasts. Methyl viologen action. J. Photochem. Photobiol. B: Biol. 12: 375-387.
Hausladen, A. and Alscher, R.G. 1993. Glutathione In: Antioxidants in Higher Plants. R.G. Alscher and J.L. Hess (eds) CRC Press, Boca Raton. pp. 1-30.
Hell, R. and Bergmann, L. 1990. Glutamylcysteine synthetase in higher plants: catalytic properties and subcellular localization. Planta 180:603-612.
Hell, R. and Bergmann, L. 1988. Glutathione synthetase in tobacco suspension cultures: catalytic properties and localization. Physiol. Plant 72:70-76.
Hertwig, B., Steb, P. and Feierabend, J. 1992. Light dependence of catalase synthesis and degradation in leaves and the influence of interferring stress conditions. Plant Physiol. 100:1547-1553.
Hess, J.L. 1993. Vitamin E, a-tocopherol. In: Antioxidants in Higher Plants. R.G. Alscher and J.L. Hess (eds) CRC Press. Boca Raton, pp.111-134.
Hohn, D.C. and Lehere, R.L. 1975. NADPH oxidase deficiency in X-linked chronic granulomatous disease. J. Clin Invest. 53:707-713.
Imlay, J.A. and Linn, S. 1986. DNA damage and oxygen radical toxicity. Science 240:1302-1309.
Jacobs, J.M., Jacobs, N.J., Sherman, T.D. and Duke, S.O. 1991. Effect of diphenyl ether herbicides in oxidation of photoporphyrinogen to protoporphyrin in organellar and plasma membrane enriched fractions of barley. Plant Physiol. 97:197-203.
Johnson, W.O., Kollman, G.E., Swithenbank, C. and Yih, R.Y. 1978. RH-6201 (Blazer): A new broad spectrum herbicide for postemergence use in soybeans. J. Agric. Food Chem. 26:285-286.
Kelner, M.J. and Bagnell, R. 1990. Glutathione-dependent enzymes alone can produce paraquat resistance. Free Rad. Biol. Med. 9:149-153.
Kendall, E.J. and McKersie, B.D. 1989. Free radical and freezing injury to cell membranes of winter wheat. Physiol. Plant. 76:86-94.
Kirkman, H.N., Galiano, S. and Gaetani. 1987. The function of catalase-bound NADPH. J. Biol. Chem. 262:660-666.
Klapheck, S. 1988. Homoglutathione: isolation, quantification and occurrence in legumes. Physiol. Plant 74:727-732.
Kouiji, H., Masuda, T. and Matsunaka, S. 1989. Action mechanism of diphenyl ether herbicides: stimulation of 5-aminolevulinic acid synthesizing system activity. Pestic. Biochem. Physiol. 33:230-238.
Kuroda,H., Sagisaka, S., Asada, M. and Chiba, K. 1991. Peroxide-scavenging systems during cold acclimation of apple callus in culture. Plant and Cell Physiology. 32:635-641.
Lehnen, L.P. Jr., Sherman, T.D., Becerril, J.M. and Duke, S.O. 1990. Tissue and cellular localization of acifluorfen-induced porphyrins in cucumber cotyledons. Pestic. Biochem. Physiol 37:239-248.
Leshem, Y.Y., 1992. Plant Membranes: A Biophysical Approach to Structure, Development and Senescence. Kluwer Academic Publishers Dordrecht, The Netherlands, 266 p.
Lindqvist, Y., Branden, C.L., Mathews, F.S., and Lederer, F. 1991. Spinach glycolate oxidase and yeast flavocytochrome b2 are structurally homologous and evolutionarily related enzymes with distinctly different function and flavin mononucleotide binding. J. Biol. Chem. 266:3198-3207.
Loewus, F.A. 1988. Ascorbic acid and its metabolic products. In: The Biochemistry of Plants, Vol. 14. Preiss, J. (ed) Academic Press, New York, p. 85-107.
Loschen, G., Azzi, A. and Floheßp, L. 1973. Mitochondrial H2O2 formation: Relationship with energy conversion. FEBS Lett 33:84-88.
Loschen, G., Azzi, A., Richter, C. and Floheßp, L. 1974. Superoxide radicals as precursors of mitochondrial hydrogen peroxide. FEBS Lett 42:68-72.
Malan, C., Greyling, M.M. and Gressel, J. 1990. Correlation between Cu/Zn superoxide dismutase and glutathione reductase, and environmental and xenobiotic stress tolerance in maize inbreds. Plant Sci. 69:157-166.
Mann, T., and Kleilin, D. 1938. Homocuprein and heptacuprein, copper-protein compounds of blood and liver in mammals. Proc. R. Soc. London B. 126:303-315.
Marx, J.L. 1985. Oxygen free radicals linked to many diseases. Science. 235-529-531.
Mathis, P. and Kleo, J. 1973. The triplet state of ß-carotene and of analog polyenes of different length. Photochem. Photobiol. 18:343-346.
Matringe, M., Camadro, J-M., Labbe, P. and Scalla, R. 1989. Protoporphyrinogen oxidase as a molecular target for diphenyl ether herbicides. Biochem J. 260:231-235.
Matsumoto, H. and Duke, S.O. 1990. Acifluorfen-methyl effects on porphyrin synthesis in Lemna pousicostata. Hegelm. 6746. J. Agr. Food Chem. 38:2066-2071.
Mayasich, J.M., Nandihalli, U.B., Liebl, R.A. and Rebeiz, C.A. 1990. The primary mode of action of acifluorfen-Na in intact seedligns is not via tetrapyrrole accumulation during the first dark period following treatment. Pestic. Biochem. Physiol. 36:259-268.
McCord, J.M. and Fridovich, I. 1969. Superoxide dismutase, an enzymatic function for erythrocuprein. J. Biol. Chem. 244:6049-6055.
McKersie, B.D., Hoekstra, F., and Krieg, L. 1990. Differences in the susceptibility of plant membrane lipids to peroxidation. Biochim. Biophys. Acta. 1030:119-126.
McKersie, B.D., Chen, Y., de Beus, M., Bowley, S.R., Bowler, C., Inzé, D., D’Halluin, K. and Boterman, J. 1993. Superoxide dismutase enhances toleance of freezing stress in transgenic alfalfa (Medicago sativa L.). Plant Physiol. (in press).
Mees, G.C. 1960. Experiments on the herbicidal action of 1,l’-ethylene-2,2′-dipyridylium dibromide. Ann. Appl. Biol. 48:601-612.
Meister, A. 1988. Glutathione metabolism and its selective modification. J. Biol. Chem. 263:17205-17208.
Morré, D.J., Brightman, A.O., Wu, L.Y., Barr, R. Leak, B. and Crane, F.L. 1988. Role of plasma membrane redox activities in elongation growth in plants. Physiol. Plant 73: 187-193.
Oleinick, N.L., Chiu, S., Ramakrishman N. and Xue, L. 1986. The formation, identification, and significance of DNA-protein cross-links in mammalian cells. Brit. J. Cancer 55: Suppl. 8:135-140.
Pallett, K.E, and Young, A.J. 1993. Caratenoids. pp. 91-110. In: Eds. R.G. Alscher and J.L. Hess. Antioxidants in Higher Plants. CRC Press, Boca Raton.
Polle, A., Chakrabarti, K., Schürmann, W., and Rennenberg, H. 1990. Composition and properties of hydrogen peroxide decomposing systems in extracellular and total extracts from needles of Norway spruce (Picea abies L., karst). Plant Physiol. 94:312-319.
Preston, C., Holtum, J.A.M. and Powles, S.B. 1992. On the mechanism of resistance to paraquat in Hordeum glaucum and H. leporium. Plant Physiol. 100:630-636.
Price, A., Lucas, P.W. and Lea, P.J. 1990. Age dependent damage and glutathione metabolism in ozone fumigated barley: a leaf section approach. J. Exptl. Bot. 41:1309-1317.
Rebeiz, C.A., Reddy, K.N., Nandihalli, U.B. and Velu, J. 1990. Tetrapyrole-dependent photosythetic herbicides. Photochem. Photobiol. 52:1099-1117.
Redinbaugh, M.G., Wadsworth, G.J. and Scandalias, J.G. 1988. Characterization of catalase transcripts and their differential expression in maize. Biochim. Biophys. Acta. 951:104-116.
Rennenberg, H. 1982. Glutathione metabolism and possible biological roles in higher plants. Phytochem. 21:2771-2781.
Rich, P.R., Bonner, W.D. Jr. 1978. The sites of superoxide anion generation in higher plant mitochondria. Arch. Biochem. Biophys. 188:206-213.
Rüegsegger, A., Schmutz, D. and Brunold, C. 1990. Regulation of glutathione biosynthesis by cadmium in Pisum sativum L. Plant Physiol. 93:1579-1584.
Sandalio, M. and Del Rio, L.A. 1988. Intraorganellar distribution of superoxide dismutase in plant peroxisomes (glyoxysomes and leaf peroxisomes). Plant Physiol. 88:1215-1218.
Scandalias, J.G. 1990. Response of plant antioxidant defense genes to environmental stress. Adv. Genet. 28:1-41.
Scandalias, J.G. 1993. Oxygen stress and superoxide dismutase. Plant Physiol. 101:7-12.
Schmidt. A., and Kunert, K.J. 1986. Lipid peroxidation in higher plants. The role of glutathione reductose. Plant Physiol 82:700-702.
Shaaltiel, Y. and Gressel, J. 1986. Multienzyme oxygen radical detoxifying system correlated with paraquat resistance in Conyza bonariensis. Pestic. Biochem. Physiol. 26:22-28.
Smith, I.K., Kendall, A.C., Keys, A.J., Turner, J.C. and Lea, P.J. 1985. The regulation of the biosynthesis of glutathione in leaves of barley (Hordeum vulgare L.). Plant Sci. 41:11-17.
Smith, I.K., Vierheller, T.L. and Thorne, C.A. 1989. Properties and functions of glutathione reductase in plants. Physiol. Plant. 77:449-456.
Smith, J. and Shrift, A. 1979. Phytogenetic distribution of glutathione peroxidase. Comp. Biochem. Physiol. 63B:39-44.
Stadtman, E.R. 1986. Oxidation of proteins by mixed-function oxidation systems: implication in protein turnover, aging and neutrophil function. Trends Biochem. Sci. 11:11-12.
Steinkamp, R. and Rennenberg, H. 1984. Glutamyltranspeptidase in tobacco suspension cultures: catalytic properties and subcellular localization. Physiol. Plant. 61:251-256.
Tepperman, J.M. and Dunsmuir, P. 1990. Transformed plants with elevated level of chloroplastic SOD are not more resistant to superoxide toxicity. Plant Mol. Biol. 14:501-511.
Timmerman, K.P. 1989. Molecular characterization of corn glutathione-S-transferase isozymes involved in herbicide detoxification. Physiol. Plant. 77:465-471.
Turrens, J.F., Freeman, B.A., Crapo, J.D. 1982. Hyperoxia increases H2O2 release by lung mitochondria and microsomes. Arch. Biochem. Biophys. 217:411-421.
Van Camp W., van Montagu M., Inze D. 1994. Superoxide dismutases. In: C. H. Foyer and P. M. Mullineaux (eds). Causes of photooxidative stress and amelioration of defense systems in plants. CRC Press Inc. Boca Raton; USA. 317-341
Vaughn, K.C. and Fuerst, E.P. 1985. Structural and physiological studies of paraquat-resistant Conyza. Pestic. Biochem. Physiol. 24:86-94.
Vianello, A., Macri, F. 1991. Generation of superoxide anion and hydrogen peroxide at surface of plant cells. J. Bioenerg. Biomemb. 23:409-423.
Wilson, D.O. and McDonald, M.B. Jr. 1986. The lipid peroxidation model of seed aging. Seed Sci. Tech. 14:269-300.
Winston, G.W., Cederbaum, A.I. 1983a. Oxyradical production by purified components of the liver microsomal mixed-function oxidase system I: Oxidation of hydroxyl radical scavenging agents. J. Biol Chem 258:1508-1513.
Witkowski, D.A. and Halling, B.P. 1989. Inhibition of plant protoporphyrinogen oxidase by the herbicide acifluorfen-methyl. Plant Physiol. 90:1239-1242.
Young, A.J. 1991. Inhibition of carotenoid biosyntheses. pp.131-171. In: Eds. N.R. Baker and M.P. Percival. Herbicides. Elsevier Publishers.
Young, A.J. 1991. The photoprotective role of carotenoids in higher plants. Physiol. Plant. 83:702-708.
Bryan D. McKersie
Dept of Crop Science, University of Guelph (December, 1996)